Research Interests
My research interests are in the fields of functional morphology and ecological physiology. My focus is in the study of the dynamics of locomotion in animals. I examine the energetics and hydrodynamics of vertebrate swimming, with particular regard to propulsive modes and the evolution of aquatic mammals. This research is accomplished by examination of morphological structures with computer tomography (CT scans), biomechanics with motion analysis and computer digitizing, and exercise physiology by measurement of metabolic performance with oxygen consumption. Research in the Liquid Life Lab has allowed my students and me to work with a variety of animals, including whales, dolphins, seals, sea lions, mantees, otters, platypus, muskrat, beaver, opossums, hippopotamus, frogfish, flying fish, sharks, mallard ducks, alligators, batoid rays and whirligig beetles. The research has been funded by the National Science Foundation (NSF), Office of Naval Research (ONR), and Defense Advanced Research Projects Agency (DARPA). The research has application in the field of biomimetics and bioinspiration of engineered systems. I served on the editorial board of the journal Bioinspiration and Biomimetics, which is published by the Institute of Physics. I am an associate editor for the journal Marine Mammal Science.
I have been profiled for my research in the book At the Water's Edge: Macroevolution and the Transformation of Life by Carl Zimmer and in the article Biomimetics: Design by Nature in the April 2008 issue of National Geographic. I have appeared on television in the PBS series Evolution, the Canadian Broadcasting Company production The Daily Planet Goes Green, the BBC production of Walking with Prehistoric Beasts, which aired on the Discovery Channel, Predator X, which aired on the History Channel, Hooked: Flying Monsters, which aired on the National Geographic Channel, the BBC production Bang Goes the Theory, and Xploration Earth 2050 - Bio-Mechanimals, which aired on Fox.
I have presented research seminars at a variety of locations, including Adelphi University, the American Museum of Natural History, Australian Institute of Sport, Brigham Young University, Brown University, Chestnut Hill Academy, Clark University, Clarkson University, Clemson University, Cornell University, Duke University, Eastern University, Flinders University of South Australia, Florida State University, Franklin and Marshall College, George Mason University, George Washington University, Georgia Institute of Technology, Hamilton College, Harvard University, Interuniversity Institute of Marine Sciences (Eilat, Israel), Johns Hopkins Hospital, Johns Hopkins University, La Trobe University, Lehigh University, Millersville University, Monterey Bay Aquarium, National Aquarium (Baltimore, MD), National Museum of Natural History (Smithsonian Institution, Washington, DC), National Zoological Park, Smithsonian Institution, NOAA Fisheries Service (LaJolla, CA), Old Dominion University, Philadelphia Academy of Natural Sciences, Philadelphia Zoo, Princeton University, Rensselaer Polytechnic Institute, Richard Stockton College of New Jersey, Saint Joseph's University, Shippensburg University, SPAWAR Systems Center (San Diego), Stanford University, Syracuse University, Swarthmore College, The Pennsylvania State University, Technion (Isreali Institute of Technology), Tokai University Pacific Center (Honolulu, Hawaii), University of Adelaide, University of California at Berkeley, University of California at Santa Cruz, University of Chicago, University of Cincinnati, University of Delaware, University of Maryland, University of Minnesota, University of North Carolina Wilmington, University of Otago, University of Pennsylvania, University of Pennsylvania Veterinary School, University of Rhode Island, University of Richmond, University of Rochester, University of San Diego, University of Scranton, University of Virginia, Vassar College, Villanova University, and Widener University.
Research Topics
Thermoregulation in aquatic animals
The aquatic environment presents challenges to animals that control their body temperture. Water is thermally more conductive than air at the same temperature. To maintain a body temperature above the temperature of the water, aquatic animals must either increase their metabolism, increase the insulation of the body, or find new sources of thermal energy.
My Master's thesis research examined the changes in insulation associated with the regulation of metabolism in the muskrat (Ondatra zibethicus). The fur of the muskrat insulates the body, but cannot be regulated. By using regional heterothermia, the muskrat could regulate the temperature of the appendages (feet, tail), with their high surface-to volume ratio, and adjust its thermal insulation. When heat needs to be conserved and insulation increased, blood flow to the appendages is reduced and the appendage takes on the temperature of the environment. In water, the muskrat allowed its appendages to approach the temperature of the medium. In air at high temperatures, the appendages were warmed once the core body temperature reached a critical level. The temperature of the appendages cycled with the core temperature.
Fish, F. E. 1979. Thermoregulation in the muskrat (Ondatra zibethicus): The use of regional heterothermia. Comparative Biochemistry and Physiology 64A(3): 391-397. pdf
How alligators thermoregulate in the water was an interesting problem that came from observations in the field and in the laboratory. In the field, alligator are seen to be floating like logs with the dorsum exposed, but in the laboratory, alligators float with the body submerged (figure A below). When a heat lamp was turned on and situtated over an insulated tank with the water temperature controlled, the alligators assumed the posture observed in the field (figure B below). These observations demonstrated that alligators will bask to warm themselves even in water.
Jet Propulsion
Locomotion in animals by jet propulsion is typically associated with the pulsing movements of the jellyfish or the rapid movements of squid and octopus. Jetting is less common in vertebrates, but is used by some species of fishes. The frogfishes (Genus Antennarius) has restricted opercular openings in the axilla of the pectoral fins. As the fish breathes, it can expel water from the opercular openings as jets. The frogfish can use the jets to take-off from the bottom or to move through the water (below). Due to the high drag of the body, the fish jets at low speeds of 2.3-2.7 cm/sec (0.27-0.32 body lengths/sec). Because the jets are located under the center of gravity of the body, the head of the fish pitches up and down, during expiration and inspiration, respectively. Jetting may be a strategy by the frogfish to sneak up on its prey. Frogfishes mimic sponges and rocks. When the frogfish gets close enough to its prey, it waves the first dorsal fin spine, which has a lure on its end. Once the prey approaches, the frogfish can rapidly engulf the prey.
Fish, F. E. 1987. Kinematics and power output of jet propulsion by the frogfish genus Antennarius(Lophiiformes: Antennariidae). Copeia 1987(4): 1046-1048. pdf
Stability and Maneuverability
The morphological designs of animals represent a balance between stability for efficient locomotion and instability associated with maneuverability. Morphologies that deviate from designs associated with stability are highly maneuverable . Major features affecting maneuverability are positions of control surfaces, control of buoyancy, and flexibility of the body. For marine mammals, variation in body design affects stability and turning performance.
Sirenians, such as manatees, have a high degree of hydrostatic control over buoyancy and thus are able to control stability without flow induced lift forces to stabilize body orientation and with a minimum of control surfaces (i.e., flippers, flukes). Cetaceans have a number of control surfaces (i.e., flippers, flukes, dorsal fin, caudal peduncle) and relatively rigid body that provides a generally stable design. Destabilizing forces generated during swimming are balanced by dynamic stabilization due to the phase relationships of various body components. Cetaceans with flexible bodies and mobile flippers (Dephinapterus, Inia) are able to turn tightly, although at low turning rates, compared to fast-swimming cetaceans (Lagenorhynchus, Cephalorhynchus, Orcinus) with less body flexibility and relatively immobile flippers. This latter group sacrifices small turning radii for higher turning rates. In cetaceans, turning performance and the morphology and placement of control surfaces are associated with prey type and habitat. Flexibility and slow, precise maneuvering are found in cetaceans that inhabit more complex habitats (i.e., rivers, pack ice), whereas high-speed maneuvers are used by cetaceans in the more open pelagic environment. As smaller prey species, such as fish, can produce smaller radius turns at higher turning rates than dolphins, these fast-swimming dolphins compensate by working in cooperative groups or by using maneuvers in which they orient the body to easily bend and bring the mouth toward the fish rapidly.
Aerial spinning is performed by spinner dolphins (Stenella longirostris). These maneuvers are spectacular leaps from the water, while rotating around the dolphin's longitudinal axis up to seven times with an airborne time of 1.25 seconds. Dolphins can reach a height of 3 meters and can perform 14 successive spinning leaps. Although twisting of the body while airborne has been proposed as the mechanism to produce the spin, the morphology of the dolphin precludes this mechanism for the spinning maneuver. The angular momentum to induce the spin is generated underwater, prior to the leap. Subsurface corkscrewing motion represents a balance between drive torques generated by the flukes and by hydrodynamic forces at the pectoral fins, and resistive torques, induced by the drag forces acting on the rotating control surfaces. As the dolphin leaps clear of the water, this balance no longer is maintained as the density of the air is essentially negligible and a net driving torque remains, which permits the dolphin’s rotation speed to increase by as much as a factor of three for a typical specimen. The high rotation rates and orientation of the dolphin’s body during re-entry into the water could produce enough force to hydrodynamically dislodge unwanted remoras. The remoras can be irritating to the sensitive skin of the dolphin.
Hawaiian spinner dolphin performing an aerial spinning leap. The dolphin has two remoras
on its skin.
The remoras on the spinner dolphins are thrown out radially due to the high rotation
rate.
Spinner dolphins showing streamlined form.
Compared to dolphins, like the bottlenose dolphin, spinner dolphins are thinner, which aids in their spinning by reducing the moment of inertia for a faster spin rate. The ability to spin is also used in feeding. Animals such as crocodilians will grasp large prey with their jaws and roll to dismember the prey. This is the much feared "Death Roll". An alligator performs the Death Roll by angling the tail away for the body axis to create the torques required for the spinning maneuver. This spinning action is described as a Zero Angular Momentum Maneuver.
Evolution and Energetics of Swimming Modes
For secondarily aquatic vertebrates, the movement from the terrestrial environment back into the water was a major evolutionary change. With approximately 70% of the planet's surface covered by water, it is not surprising that a wide variety of mammalian lineages have secondarily invaded the aquatic environment. However, moving into the viscous medium of water, where Archimedes Principle dominates, from the less-viscous, gravity-dominated terrestrial environment has presented biomechanical and energetic hurdles to these mammals.
Those animals in the most energetically precarious position are semiaquatic mammals. Semiaquatic mammals occupy the intermediate position between terrestrial and aquatic animals, in which they are not specialized for either environment. Their energetic performance in each environment is limited by an anatomy and physiology which are compromises to the disparate forces experienced on land and in water. With the increased aquatic habits, propulsive appendages of semiaquatic mammals were modified only slightly from the weight-bearing, inverted pendular struts necessary for terrestrial locomotion. Despite the aquatic habits of these intermediate forms, it should also be remembered that these animals are semi-terrestrial as well as semiaquatic. Therefore, it is expected that as the morphology changes with increasing aquatic habits, then their energetic efficiency decreases for locomotion on land.
Whereas swimming by semiaquatic mammals is associated with inefficiencies and high energetic costs, the converse is considered true for aquatic mammals. Aquatic mammals are far removed from their terrestrial ancestors and as such are specialized to live and forage primarily in water. In this regard, evolution of the propulsive appendages has shaped these structures into high efficiency hydrofoils which effect locomotion by transferring momentum to fluid surroundings. This change in limb structure, although negatively impacting an aquatic mammal's performance on land, enhanced the energetic performance in the water.
Despite these apparent differences, all lineages of aquatic mammals had terrestrial ancestors and thus underwent a semiaquatic phase. The evolution of increased aquatic habits in many mammals necessitated the evolution of adaptations that allowed aquatic mammals to optimize energy use by reduction of resistive forces, improvement in propulsive force production and efficiency, thermoregulation, and control of buoyancy. Because modern semiaquatic mammals are the best representations of the transitional aquatic forms, direct comparison of energetics can point to the potential selective factors and mechanical constraints that directed the evolution of more derived aquatic forms.
Conceptional model diagraming the sequence of swimming mode changes in the evolution of aquatic mammals. The end-points are represented by phocid seals (pelvic oscillation), cetaceans and sirenians (caudal oscillation), and otarid seals (pectoral oscillation). Major transitions in swimming modes occurred with diving activiities and changes insulation from fur to blubber.
Animals that are semiaquatic are also semiterrestrial. As they are not specialized to either environment, there are increased energetics costs of locomotion in either environment. As animals become more aquatic, they expend more energy to move on land than in water as shown in the graph above. The increased energetic cost is associated with morphological changes that make the animal more effective in water but less able to move about on land, such as the possession of flippers on the phocid seal to the right. The transition from terrestrial to aquatic vertebrates required that high energy resources were available as these animals invaded the new habitat.
In the case of the Northern elephant seal, the massive pinniped must be able to swim in the water with its flippers, but still be able to move on land. They are able to perform terrestrial locomotion by using a traveling spinal wave that moves anteriorly along the dorsal margin of the body with the chest, pelvic region, and foreflippers serving as the main points of contact with the ground. The fore flippers are used as crutches to lift the anterior of the body as the animal is pushed forward from the pelvic region that remains in contact with the ground. The hind flippers are not used in terrestrial locomotion. Elephant seals expend more energy when traveling over land for their size than smaller phocids.
Three-Dimensional Geometry of Biological Control Surfaces
The immense diversity of animals with their particular morphological features presents a rich resource of novel designs that may be incorporated into advanced technologies. The technology associated with the development of robots is becoming more dependent on biomimetics and biologically-inspired designs. The morphology of animals have been copied for development of various technologies. Both machines and animals must contend with the same physical laws that regulate their design and behavior. These behaviors (i.e., maneuverability, acceleration) can be superior to the performance of machines.
The control surfaces of animals have different functions including propulsion, maneuverability, braking, trim control, hovering, reverse swimming, and stability. In nature, the control surfaces are used for prey capture, prey acquisition, escape maneuvers, obstacle avoidance, turning in restricted spaces, surfacing, diving, and control of rapid accelerations. Stealth may be an important characteristic of maneuvering with pectoral fins as prey acquisition by a predator often requires approach without detection. Various morphologies within aquatic animal lineages have evolved which foster maneuverability. Turning performance can be affected by morphology with respect to rigidity of the body, and mobility and position of the control surfaces (e.g., fins, paddles, flippers) determining the level of performance.
Despite the importance of the control surfaces, there has been little information on the structure, function, and hydrodynamics of aquatic biological control surfaces. A description of the morphological variation and three-dimensional geometry of the control surfaces of nektonic organisms would be beneficial to both biologist and engineers.
My research on the control surfaces has used medical computer tomography (CT) scans to examine the three-dimensional geometry for a variety of vertebrate species, including dolphins, whales, porpoises, manatee, sea lion, seals, penguins, sea turtles, tuna, sharks and rays.
Bio-inspiration and Biomimetics
The incorporation of novel structures and mechanisms from nature into the design and function of machines is being attempted through biomimetics. Biomimetics, or what was previous called bionics, attempts to produce engineered systems that possess characteristics, resemble, or function like living systems. The goal of biomimetics in the field of robotics is to use biological inspiration to engineer machines that emulate the performance of animals, particularly in instances where the animal’s performance exceeds current mechanical technology.
It has been a long standing idea that new technologies can be developed from natural. Animals have served as the inspiration for various technological developments. Copying animals by the biomimetic approach attempts to seek common solutions from engineering and biology for increased efficiency and specialization. Because biological designs resulted from the evolutionary Darwinian process of "natural selection", it is considered that animals have already performed the “cost-benefit-analysis”, optimizing particular designs for specific functions. The diverse morphological specializations exhibited by animals may be targeted by engineers for technology transfer and effectively reduce the time of development of innovative technological solutions.
Differences between engineered systems and animal systems are apparent. Engineered systems are relatively large in size, are composed of rigid materials, use rotation motors, and are controlled by computational systems that have limited sensory feedback; whereas, animals are generally small in size, are composed of compliant materials, use translational movements produced by muscles, and are controlled by complex neural networks with multiple sensory inputs. In addition, animals are functionally multifaceted (i.e., they move, feed, and reproduce) and must compromise optimal solutions for specialized functions to perform adequately rather than maximally.
The humpback whale has pronounced bumps or tubercles along the leading edge of its pectoral flippers (above left). A idealized model of the flipper (above upper right), which was tested in a wind tunnel, demonstrated that the tubercles enhanced hydrodynamic performance, particularly by delaying stall (i.e., dramatic loss of lift) of the flipper with increasing angle of attack. The tubercles were placed on biomimetic windmill blades (above lower right). Application of the tubercle technology has been taken up for use in wind turbines, fans, pumps and compressors, and surfboard skegs.
Another application of the humpback whale tubercles is on control surfaces (e.g., rudders, keels, dive planes) and wings. A human-powered submarine, Umpty Squash, utilized tubercled dive planes and rudders. Students of the Sussex County Technical High School, Sparta, NJ, constructed the submarine. In 2005, the submarine competed in the International Submarine Races held at the David Taylor Model Basin in Bethesda, Maryland. The submarine was capable of making a 90o turn within 25 feet. The 2011 version of Umpty Squash used the tubercles on the front dive planes. The submarine used a biomimetic form of propulsion in which the vehicle moved with a fish-like motion.
Umpty Squash (2005) built by the students of the Sussex County Technical High School.
Another research interest concerns aquatic propulsion by oscillating flukes as a biomimetic alternative to typical marine propellers. The flukes of cetaceans possess geometries with flexibility, which enhance thrust production for high efficiency swimming. The propuslive efficiency of the flukes of various cetacean species is higher than the efficiency of standard marine propellers.
Biomechanics of Batoid Ray Swimming
The Liquid Life Lab is collaborating with researchers from the University of Virginia, Princeton University, and UCLA to examine the swimming motions, 3D geometry, and material properties of the batoid rays as the inspiration for development of Biomimetic Autonomous Undersea Vehicles (BAUV). Particular attention is focused on the manta ray (Manta birostus, order Myliobatiformes, family Mobulidae). Rays embody many of the qualities required in an ideal underwater vehicle: the flexible nature of the propulsive surfaces and the ability to modulate flow, high-efficiency cruising ability, agile maneuverability for obstacle avoidance, ability to follow bottom terrain, and ability to hold station. In addition, these animals can function in the open ocean and are capable of precise maneuvering in the littoral region.
Atlantic stingray being tested in long tank in the Liquid Life Lab
Filming manta at the Georgia Aquarium with (left to right) Jessica Hoffman, Rachel
Nichols, and Elizabeth Barchi.
F. Fish with manta at the Georgia Aquarium, where the motions of the ray were video
recorded.
Research at Sea World Discovery Cove in Orlando, FL to video record the swimming movements
of cownose rays and spotted eagle rays with Carly Ginter and Jana Parson.
Work continues on the mechanics of swimming by batoids. A new digital particle image velocimetry (DPIV) system as been added into the Liquid Life Laboratory. With DPIV, the wake structure of a swimming ray can be visualized and measured to help determine the development of thrust. The images below show a ray swimming through a laser sheet to illuminate microscopic reflective particles in the water (Middle) and the vector flow in the wake of the ray (Right) using the La Vision system. A pronounced vortex can be observed behind the ray.
The information provided from the morphology and kinematics of swimming rays has been incorporated into the design and performance of robotic rays that were constructed at the University of Virginia and Princeton University. The kinematics of the University of Virginia MantaBot is shown above.
Video of the MantaBot swimming performance in a pool can be observed from the website: http://www.youtube.com/watch?v=d3-GbTuXazs&feature=youtu.be
In the fall of 2012, the MantaBot was brought to the WCU pool for testing and video recording.
The MantaBot was tested in the pool at a depth of eight feet, so SCUBA had to be used to set up the cameras for video recording. The MantaBot was tested for speed and maneuvering control, where the MantaBot successful Figure-8 turns.
Getting the MantaBot ready for trials from left to right were engineers Joe Zhu and Trevor Kemp from the University of Virginia, and West Chester University students Molly Gabler, Janet Fontanella, and Griffin Lewis.
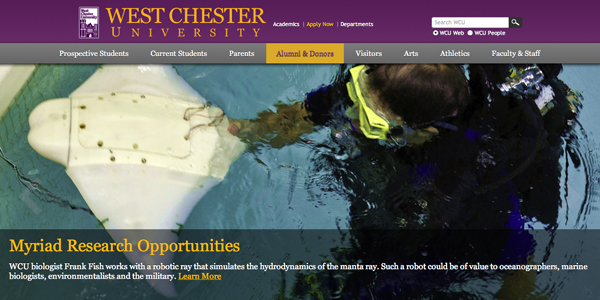
The work on the MantaBot was highlighted on the West Chester University website.
Robotic Sea lion
I have been working with George Washington University and Drexel University on an ONR-sponsored project to construct a robotic sea lion. My function is to gather data on the swimming and walking capabilities of sea lions.
Hydrodynamic Flipper Project
In an aquatic environment, the control of stability and maneuvering of animals is determined by the morphology of the control surfaces. The position, mobility, hydrodynamic characteristics of the control surfaces are associated with swimming performance. For cetaceans (whales, dolphins, porpoises), the pectoral flippers are mobile, hydrofoils. The flippers have a cross-sectional design, similar to engineered hydrofoils for lift generation. The flippers contribute to lateral turning, diving, surfacing, braking, trim control, and reduction of recoil from propulsive movements. Flippers are a modification of the pectoral appendages. They enclose a skeletal framework based on the terrestrial mammalian forelimb. Flipper shape can vary from elongate, wing-like appendages with tapering tips to short, rounded paddle-like structures. Although cetacean flippers are the quintessential example of evolutionary homology and discussed in textbooks in comparative anatomy, evolution, paleontology and functional morphology, little is known of the diversity of flipper geometry within the Cetacea and the hydrodynamic characteristics and function of these control surfaces.
In 2007, the National Science Foundation gave a grant (IOS - 0640185) to the team of Frank Fish (West Chester University), Laurens Howle (Duke University), and Mark Murray (United States Naval Academy) to investigate the three-dimensional geometry and hydrodynamic performance of cetacean flippers with differing morphologies for an integrated, collaborative approach using biological and engineering analyses.
![]() |
![]() |
![]() |
Frank Fish | Laurens Howle | Mark Murray |
The three-dimensional geometry of cetacean flippers is being analyzed from specimens
obtained from stranded cetaceans. The three-dimensional shape of the flippers is analyzed
using X-ray computer tomography (CT-scans). The two- and three-dimensional geometry
of the flippers is compared between species to assess hydrodynamic adaptation. Based
on the CT-scan-generated geometry, scale physical models of the flippers are fabricated.
The models are tested in a water tunnel at whale-appropriate Reynolds number over
a range of angles of attack (-30o to 30o) and whale-appropriate sweep angles to measure
lift and drag. The morphometric and hydrodynamic data will be combined using principal
components analysis (PCA) to define ecomorphological groups related to locomotor performance.
This study provides the first hydrodynamic analysis of cetacean flipper design. Application of engineering techniques to study biological structures will provide a greater understanding of the relationship between performance and morphology for large and hard to study aquatic animals.
This project emphasizes the union of biological and engineering approaches to answer comparative questions regarding the design of relevant hydrodynamic structures. The research will increase our understanding of the functional morphology of whales and dolphins in relation to swimming performance and ecology. Because of the large size of cetaceans, there has been precious little data relating morphology and locomotor performance. Functional morphologists interested in aquatic locomotion of animals will gain valuable insight into maneuverability at high Reynolds number and drag and lift performance in differing biological designs. This work is tied to an understanding of fluid dynamic control mechanisms. There is a strong possibility that the hydrodynamic results of the work on flipper morphology can be applied in the design of watercraft. Demonstration of significant practical applications on the association of natural morphologies and performance is likely to attract considerable interest from engineers through the biomimetic approach.
When a phenomenon in nature is mimicked for practical applications, it is often done so in an idealized fashion, such as representing the shape found in nature with convenient, piece-wise smooth mathematical functions. The aim of idealization is to capture the advantageous features of the natural phenomenon without having to exactly replicate it, and it is often assumed that the idealization process does in fact capture the relevant geometry. The consequences of the idealization process were explored by creating exact scale models of cetacean flippers using CT scans, creating corresponding idealized versions, then determining the hydrodynamic characteristics of the models via water tunnel testing. The majority of the idealized models did not exhibit fluid dynamic properties that were drastically different from those of the real models, although multiple consequences resulting from the idealization process were evident. For example, the minimum drag coefficient of the idealized models was less than the corresponding of the real models for all trials. The maximum lift coefficient for the real and corresponding idealized models was different for all trials, and was improved in some cases by the idealization process and worsened in others. Stall characteristics were sometimes varied by the idealization process, usually at lower Reynolds numbers. The principal causes of the variations in hydrodynamic performance were differences in the smoothness of the idealized and real flipper surfaces and the streamlining of the idealized flipper cross-sections using an airfoil profile.
![]() |
![]() |
||
Close up of flipper model in US Naval Academy flow tank | Lift and drag on pectoral flipper of a harbor porpoise in regard to angle of attack. | Morphology of different cetacean flippers tested. | Flipper in flow tank showing force sensor and turntable to give angle of attack. |